Utility-Scale PV
Units using capacity above represent kWAC.
2021 ATB data for utility-scale solar photovoltaics (PV) are shown above. The Base Year estimates rely on modeled capital expenditures (CAPEX) and operation and maintenance (O&M) cost estimates benchmarked with industry and historical data. Capacity factor is estimated for 10 resource classes, binned by mean global horizontal irradiance (GHI) in the United States. The 2021 ATB presents capacity factor estimates that encompass a range associated with advanced, moderate, and conservative technology innovation scenarios across the United States. Future year projections are derived from bottom-up benchmarking of PV CAPEX and bottom-up engineering analysis of O&M costs.
The three scenarios for technology innovation are:
- Conservative Technology Innovation Scenario (Conservative Scenario): lower levels of R&D investment with minimal technology advancement and current global module pricing
- Moderate Technology Innovation Scenario (Moderate Scenario): R&D investment continuing at similar levels as today, with current industry technology road maps achieved, but no substantial innovations or new technologies introduced to the market
- Advanced Technology Innovation Scenario (Advanced Scenario): an increase in R&D spending that generates substantial innovation, allowing historical rates of development to continue.
A detailed description of the scenarios is below.
Resource Categorization
The ATB provides the average capacity factor for 10 resource categories in the United States, binned by mean GHI. Average capacity factors are calculated using county-level capacity factor averages from the Renewable Energy Potential (reV) model for 1998–2019 (inclusive) of the National Solar Radiation Database (NSRDB). The NSRDB provides modeled spatiotemporal solar irradiance resource data at 4-km spatial and 0.5-hour temporal resolution. The county-level mean GHI is calculated by aggregating each individual NSRDB point’s multiyear mean GHI to provide a county’s mean GHI for all years included in the analysis. The U.S. average capacity factor for each resource category is weighted by the land area (square miles) of each county within the GHI resource category. The county estimated land area is provided by geospatial and tabular data from the U.S. Census.
The map below shows average annual GHI in the United States.
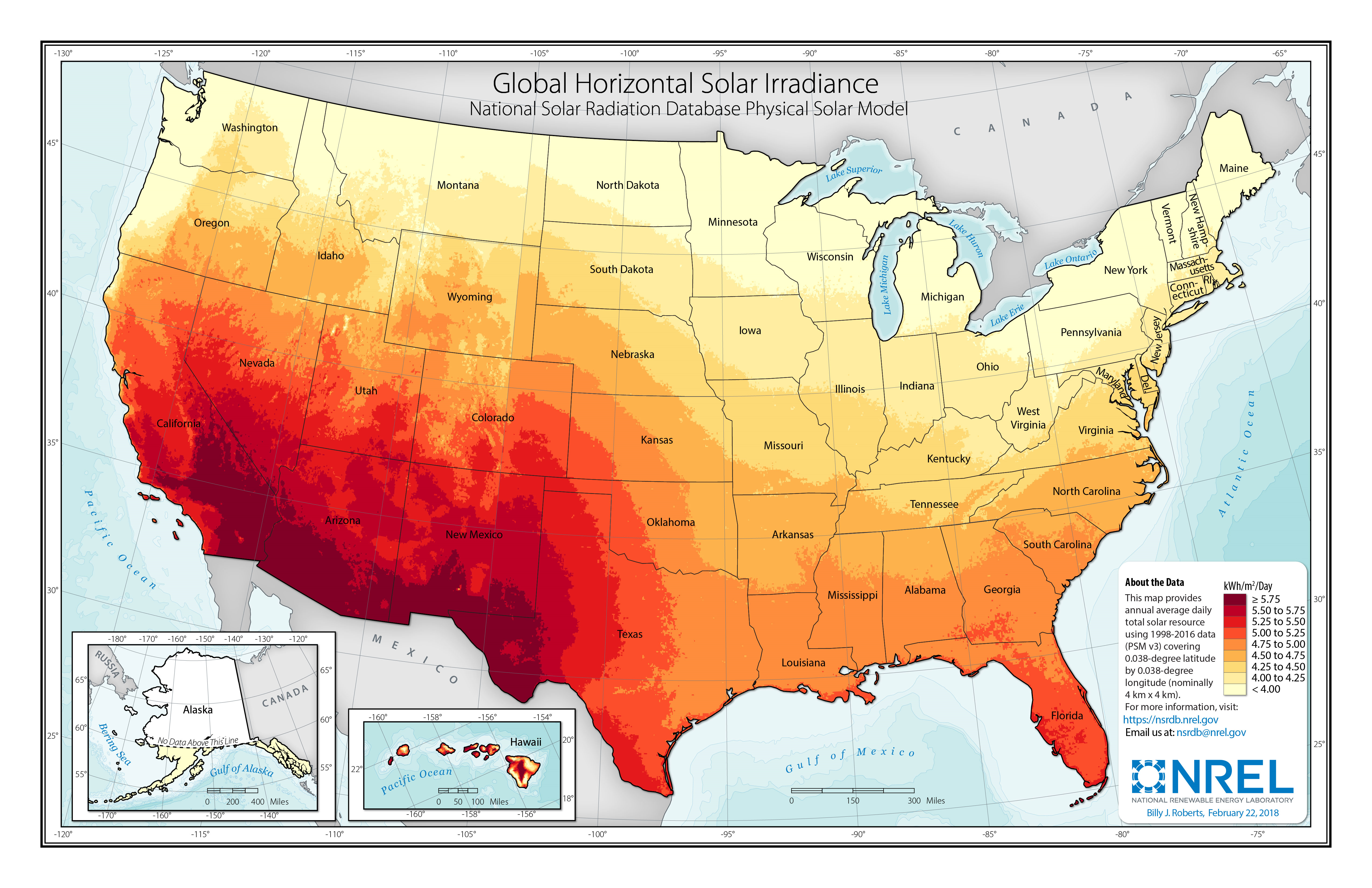
The following table summarizes the estimated 2019 capacity factors (in the first year of operation) per resource category and each resource category's associated U.S. land area.
Resource Class | GHI Bin | Mean AC Capacity Factor | Area (sq. km) |
1 | >5.75 | 32.80% | 216,551 |
2 | 5.5–5.75 | 31.80% | 349,894 |
3 | 5.25–5.5 | 30.30% | 372,764 |
4 | 5–5.25 | 28.70% | 497,444 |
5 | 4.75–5 | 26.80% | 779,720 |
6 | 4.5–4.75 | 25.80% | 870,218 |
7 | 4.25–4.5 | 24.60% | 727,918 |
8 | 4–4.25 | 23.40% | 828,438 |
9 | 3.75–4 | 22.30% | 794,496 |
10 | <3.75 | 20.40% | 163,120 |
DOE’s Solar Energy Technologies Office sets its PV cost targets for a location centered geographically within the continental U.S., in resource class 7, whereas the ATB benchmark is class 5, representing the national-average solar resource.
Scenario Descriptions
Scenario | Module Efficiency1 | Inverter Power Electronics | Installation Efficiencies | Energy Yield Gain1 |
---|---|---|---|---|
Conservative Scenario | Technology Description: Tariffs on PV modules expire, as scheduled, though some form of friction still remains, keeping U.S. panel pricing halfway between current U.S. and global pricing. Efficiency gains for panels are consistent with one standard deviation below that of the International Technology Roadmap for Photovoltaic (ITRPV—an annual report prepared by many leading international poly-Si producers, wafer suppliers, c-Si solar cell manufacturers, module manufacturers, PV equipment suppliers, and production material providers, as well as PV research institutes and consultants) to 2030, which is well below historical monofacial average gains and is below the leveling-off point to 21.5% by 2030, resulting in a price of $0.32/WDC Justification: This scenario represents the low end of industry expectations of average module efficiency in 2030 and additional friction despite the scheduled removal of the tariff. | N/A | N/A | N/A |
Moderate Scenario | Technology Description: Tariffs expire, as scheduled, and efficiency gains are consistent with median the ITRPV road map to 2030, which are well below historical monofacial average gains and are below the leveling-off point to 22.5% by 2030, which results in a price of $0.19/WDC Justification: This scenario represents manufacturers' expectations for 2030. | Technology Description: This scenario assumes medium-voltage power transmission or centralized power conversion center for inverters of $0.04/WDC. Justification: Industry is currently switching to this practice. | Technology Description: This scenario includes 30% labor and hardware balance-of-system (BOS) cost improvements through automation, preassembly efficiencies (e.g. module mounting, and wiring), and improvements in wind load design Justification: This scenario represents lower levels of improvement than the historical average (Feldman et al., 2021). With increased global deployment and a more efficient supply chain, preassembly of module mounting and wiring is possible. Best practices for permitting interconnection and PV installation (e.g. subdivision regulations, new construction guidelines, and design requirements) are being developed. | Technology Description: This scenario assumes a 5% energy gain through lower system losses, increased use of bifacial modules, improvements in bifaciality, and a degradation rate reduction from 0.7%/yr to 0.5%/yr Justification: Significant R&D is currently spent on better tracking, improved cell temperatures and lower degradation rates. Companies will likely continue to focus on improved uptime to maximize profitability, and bifacial modules are already becoming a significant part of the global and U.S. supply chain. ITRPV estimates bifacial modules' world market share will grow from 10% in 2018 to over 60% by 2030. Industry participants have already demonstrated bifacial energy gains of 5%–33%, depending on the module mounting and other factors like albedo. |
Advanced Scenario | Technology Description: Modules maintain the historical average of 0.5% improvement per year to 25% by 2030, which results in a price of $0.17/WDC Justification: Manufacturers reported mass produced cell efficiencies will increase from 20%–23% in 2018 to 21%–24% by 2021. Mass produced-monocrystalline and silicon heterojunction have already achieved cell efficiency records in a laboratory of 26.1% and 26.7% respectively.
| Technology Description: This scenario assumes inverter design simplification and manufacturing automation results in an inverter price of $0.03/WDC. Justification: The power electronics industry already has road maps to simplify and automate current products and there is more potential with increased industry size. | Technology Description: This scenario assumes 40% labor and hardware BOS cost improvements through automation and preassembly efficiencies (e.g. module mounting, and wiring); the use of carbon fiber, which is assumed to have achieved low-cost, replacing steel and aluminum, cuts mounting costs. Justification: This scenario represents lower levels of improvement than the historical average (Feldman et al., 2021). With increased global deployment and a more efficient supply chain, preassembly of PV module mounting and wiring is possible. Reduction of supply chain margins (e.g., profit and overhead charged by suppliers, manufacturers, distributors, and retailers), will likely occur naturally as the U.S. PV industry grows and matures. Additionally, streamlining of installation practices through improved workforce development and training and developing standardized PV hardware is assumed. | Technology Description: This scenario assumes a 20% energy gain through lower system losses, increased use of bifacial modules, improvements in bifaciality, and improved siting to increase average albedo from 0.2 to 0.3 without a significant increase to site prep costs (which is similar to dirt, gravel, and concrete), and a degradation rate reduction from 0.7%/yr to 0.2%/yr Justification: In addition to the justifications listed above, industry participants have already demonstrated bifacial energy gains of 5%–33%, depending on the module mounting, location, and albedo. |
General Impacts |
|
|
|
|
References |
1 Module efficiency improvements represent an increase in energy production over the same area of space, in this case, the dimensions of a PV module. Energy yield gain represents an improvement in capacity factor, relative to the rated capacity of a PV systems. In the case of bifacial modules, the increase in energy production between two modules with the same dimensions does not currently change the capacity rating of the module under standard test conditions, as the rating is based on light from one direction. Additionally, the rated capacity of a system does not increase with fewer system losses (e.g., panel cleanings).
Representative Technology
Utility-scale PV systems in the 2021 ATB are representative of one-axis tracking systems with performance and pricing characteristics in-line with a 1.34 DC-to-AC ratio-or inverter loading ratio (ILR) for current and future years (Feldman et al., 2021). We recognize that ILR is likely to change in the future, particularly with the adoption of bifacial modules, and to also be highly dependent on location. However, allowing for this change would require the optimization of ILR and CAPEX by resource bin and year, causing a range of prices, independent of other regional factors. We believe this would create less transparency and more confusion to the impact of technology changes to these individual LCOE categories.
Methodology
This section describes the methodology to develop assumptions for CAPEX, O&M, and capacity factor. For standardized assumptions, see labor cost, regional cost variation, materials cost index, scale of industry, policies and regulations, and inflation.
PV projections in the 2021 ATB are driven primarily by CAPEX cost improvements, along with improvements in energy yield, operational cost, and cost of capital (for the Market + Policies Financial Assumptions Case).
Though CAPEX is one of the drivers of lower costs, R&D efforts continue to focus on other areas to lower the cost of energy from utility-scale PV, such as longer system lifetime and improved performance. We developed three scenarios for system cost and performance characteristics in 2030, modeling as bounding levels:
- Conservative Scenario: lower levels of R&D investment with minimal technology advancement and current global module pricing
- Moderate Scenario: R&D investment continuing at similar levels as today, with current industry technology road maps achieved, but no substantial innovations or new technologies introduced to the market
- Advanced Scenario: an increase in R&D spending that generates substantial innovation, allowing historical rates of development to continue.
Capital Expenditures (CAPEX)
Definitions: For a PV system, the rated capacity in the denominator is reported in terms of the aggregated capacity of either all its modules or all its inverters. PV modules are rated using standard test conditions and produce direct current (DC) energy; inverters convert DC energy/power to alternating current (AC) energy/power. Therefore, the capacity of a PV system is rated either in MWDC via the aggregation of all modules' rated capacities or in MWAC via the aggregation of all inverters' rated capacities. The ratio of these two capacities is referred to as the inverter loading ratio (ILR). The 2021 ATB assumes current estimates, and future projections use an inverter loading ratio of 1.34.
The PV industry typically refers to PV CAPEX in units of $/MWDC based on the aggregated module capacity. The electric utility industry typically refers to PV CAPEX in units of $/MWAC based on the aggregated inverter capacity; starting with the 2020 ATB, we use $/MWAC for utility-scale PV.
Plant costs are represented with a single estimate per innovations scenario, because CAPEX does not correlate well with solar resource.
For the 2021 ATB—and based on (EIA, 2016) and the NREL Solar PV Cost Model (Feldman et al., 2021)—the utility-scale solar PV plant envelope is defined to include items noted in the table above.
Base Year: A system price of $1.36/WAC in 2019 is based on modeled pricing for a 100-MWDC, one-axis tracking systems quoted in Q1 2019 as reported by (Feldman et al., 2021), adjusted from $/WDC to $/WAC by an ILR of 1.34. The $1.35/WAC price in 2020 is based on modeled pricing for a 100-MWDC, one-axis tracking systems quoted in Q1 2020 as reported by (Feldman et al., 2021).
We focus on larger systems for the 2019 and 2020 values to better align with current trends in utility-scale installations. (EIA, 2020) reported 92 PV installations (greater than 5 MWAC in capacity) totaling 4.4 GWAC were placed in service in 2019 in the United States. Though this represents an average of approximately 48 MWAC, 76% of the installed capacity in 2018 came from systems greater than 50 MWAC, and 39% came from systems greater than 100 MWAC.
In the chart of historical data below, reported historical utility-scale PV plant CAPEX (Bolinger et al., 2020) is shown in box-and-whiskers format for comparison to the historical benchmarked utility-scale PV plant overnight capital cost and future CAPEX projections. (Bolinger et al., 2020) provide statistical representation of CAPEX for 93% of all U.S. utility-scale PV capacity.
Historical Sources: (Bolinger et al., 2020); Feldman et al. Future Projections: 2021 ATB
All prices quoted in WDC are converted to WAC (1 WDC = ILR × WAC).
The difference in each year's price between the market and benchmark data reflects differences in methodologies. Reported and benchmark prices can differ for a variety of reasons, as outlined by Barbose and Darghouth (Barbose et al., 2019) and Bolinger, Seel, and Robson (Bolinger et al., 2019), including:
- Timing-related issues: For example, the time between PPA contract completion and project placement in service can vary, and a system can be reported as installed in separate sections over time or when an entire complex is complete. For example, in 2014, the reported capacity-weighted average system price was higher than 80% of system prices in 2014 because very large systems with multiyear construction schedules were being installed that year. Developers of these large systems negotiated contracts and installed portions of their systems when module and other costs were higher.
- Variations over time in the size, technology, installer margin, and design of systems installed in a given year
- Which cost categories are included in CAPEX (e.g., financing costs and initial O&M expenses).
Federal investment tax credits provide an incentive to include costs in the upfront CAPEX to receive a higher tax credit, and these included costs might have otherwise been reported as operating costs. The bottom-up benchmarks are more reflective of an overnight capital cost, which is in-line with the ATB methodology of inputting overnight capital cost and calculating construction financing to derive CAPEX.
Use the following table to view the components of CAPEX.
Future Years
Projections of utility-scale PV plant CAPEX for 2030 are based on bottom-up cost modeling, with a straight-line change in price in the intermediate years between 2020 and 2030. ILR is assumed to remain at a constant 1.34 over time. The system design and price changes made in the models are summarized and described in the Summary of Technology Innovations by Scenario table. See below for the details of changes to components of system price in the different ATB scenarios.
We assume each scenario's CAPEX in 2050 is the equivalent of the CAPEX in 2030 but one degree more aggressive, with a straight-line change in price in the years between 2030 and 2050. Asterisks indicate corresponding cells, where scenarios use the same values but shifted in time. We also develop and model a scenario one degree more aggressive than the Advanced Scenario to estimate its 2050 CAPEX. The Advances Scenario assumes:
- Module efficiency of 30% achieved by 2030
- Further inverter simplification and manufacturing automation
- 50% labor and hardware BOS cost improvements through automation and preassembly of module mounting and wiring efficiencies
- Carbon fiber achieves low costs, replacing steel and aluminum which cuts material costs in half.
Year | Advanced (Increased R&D) | Moderate (Current R&D) | Conservative (Decreased R&D) |
---|---|---|---|
2030 | * Utility-scale CAPEX: $0.62/WAC | † Utility-scale CAPEX: $0.76/WAC | Utility-scale CAPEX: $1.16/WAC |
2050 | $0.47/WAC | * $0.62/WAC | † $0.76/WAC |
We compare the CAPEX scenarios over time to six analysts' projections, adjusted for inflation and ILR. Analyst projections represent analyst expected outcomes, while the Advanced Scenario represents NREL's estimate of an unlikely outcome (20%-50% likelihood). The CAPEX Conservative Scenario is higher than all analysts' projections; the 2030 Moderate and Advanced Scenarios are more aggressive than many, or all, analyst projections. By 2050, the Moderate Scenario represents the upper bound of analyst projections while the Advanced Scenario represents the lower bound of analyst projections (see chart below).
Sources: 2021 ATB; (EIA, 2021); (International Energy Agency (IEA), 2020); (BNEF, 2021); (BNEF, 2020) ; (Cox, 2020)
All prices quoted in WDC are converted to WAC (1 WAC =ILR × WDC).
Operation and Maintenance (O&M) Costs
Definition: Operation and maintenance (O&M) costs represent the annual fixed expenditures required to operate and maintain a solar PV plant over its lifetime, including items noted in the table above.
Base Year: O&M of $23/kWAC – yr is based on modeled pricing for a 100-MWDC, one-axis tracking systems quoted in Q1 2019 as reported by (Feldman et al., 2021) adjusted from DC to AC. The values in the 2021 ATB are higher than those in the 2020 ATB because we include costs in this year's edition of the ATB that were not previously calculated. These include five additional line measures (land lease, property taxes, insurance, asset management, and security) that are added based on feedback collected by Lawrence Berkeley National Laboratory (LBNL) from U.S. solar industry professionals (Wiser et al., 2020).
The wide range in reported prices depends in part on the range in maintenance practices for various systems and on cost categories that include asset management (including compliance and reporting for incentive payments), insurance products, site security, cleaning, vegetation removal, and component failure. Not all these practices are performed for each system; additionally, some factors depend on the quality of the parts and construction. NREL analysts estimate O&M costs can range from $0 to $40/kWDC-yr.
Future Years: Fixed O&M (FOM) of $23/kWAC-yr for 2020 is based on pricing reported by (Feldman et al., 2021), which can be divided into system-related expenses ($13/kWAC-yr), property-related expenses ($6/kWAC-yr), and administration-related expenses ($4/kWAC-yr). From 2021 to 2050, system-related FOM is based on the historical average ratio of O&M costs ($/kW-yr) to CAPEX costs ($/kW) of 1.0:100, as reported by (Feldman et al., 2021). This ratio is higher than the ratio of O&M costs to historically reported CAPEX costs of 0.8:100, which is derived from 2011–2018 historical data reported by Bolinger, Seel, and Robson (Bolinger et al., 2019), as well as the ratio of O&M costs to CAPEX costs of 1.0:100, which is derived from IEA (IEA, 2018) and Lazard (Lazard, 2018). Historically reported data suggest O&M and CAPEX cost reductions are correlated; from 2011 to 2018, fleetwide average O&M and CAPEX costs fell 43% and 64% respectively, as reported by Bolinger, Seel, and Robson (Bolinger et al., 2019). From 2021 to 2050, property-related expenses are reduced by the inverse ratio of the increase in module efficiency, as less space will be required on a per watt basis. Administrative expenses are kept constant.
Capacity Factor
Definition: The capacity factor represents the expected annual average energy production divided by the annual energy production, assuming the plant operates at rated capacity for every hour of the year. It is intended to represent a long-term average over the lifetime of the plant; it does not represent interannual variation in energy production. Future year estimates represent the estimated annual average capacity factor over the technical lifetime of a new plant installed in a given year.
PV system inverters, which convert DC energy/power to AC energy/power, have AC capacity ratings; therefore, the capacity of a PV system is rated in MWAC, or the aggregation of all inverters' rated capacities, or MWDC, or the aggregation of all modules' rated capacities. Other technologies' capacity factors are represented exclusively in AC units; however, in previous editions of the ATB, PV pricing is represented in $/kWDC. In the 2021 ATB, utility-scale PV (though not commercial PV or residential PV) is represented in $/KWAC; for this reason, values in the 2021 ATB are not directly comparable to the 2019 ATB or earlier editions of the ATB without adjusting previous versions from WDC to WAC.
The capacity factor is influenced by the hourly solar profile, technology (e.g., thin-film or crystalline silicon), the bifaciality of the module, albedo, axis type (i.e., none, one, or two), shading, expected downtime, ILR, and inverter losses to transform from DC to AC power. The DC-to-AC ratio is a design choice that influences the capacity factor. PV plant capacity factor incorporates an assumed degradation rate of 0.7%/yr in the annual average calculation. R&D could increase energy yield through bifaciality, improved albedo, better soil removal, improved cell temperature, lower system losses, O&M practices that improve uptime, and lower degradation rates of PV plant capacity factor; future projections assume energy yield gains of 0%—25% depending on the scenario.
At the end of 2019, the cumulative capacity-weighted average AC capacity factor for all utility-scale U.S. projects installed at the time (including fixed-tilt systems) was 25.0%, but individual project-level capacity factors exhibited a wide range (14%–35%). The reported U.S. system capacity factors are consistent with the range of estimated capacity factors in the 2021 ATB (19%—31%) and the calculated U.S. area-weighted 30-year lifetime average capacity factor (24.5%). The figure below shows historical data for capacity factor as a function of ILR.
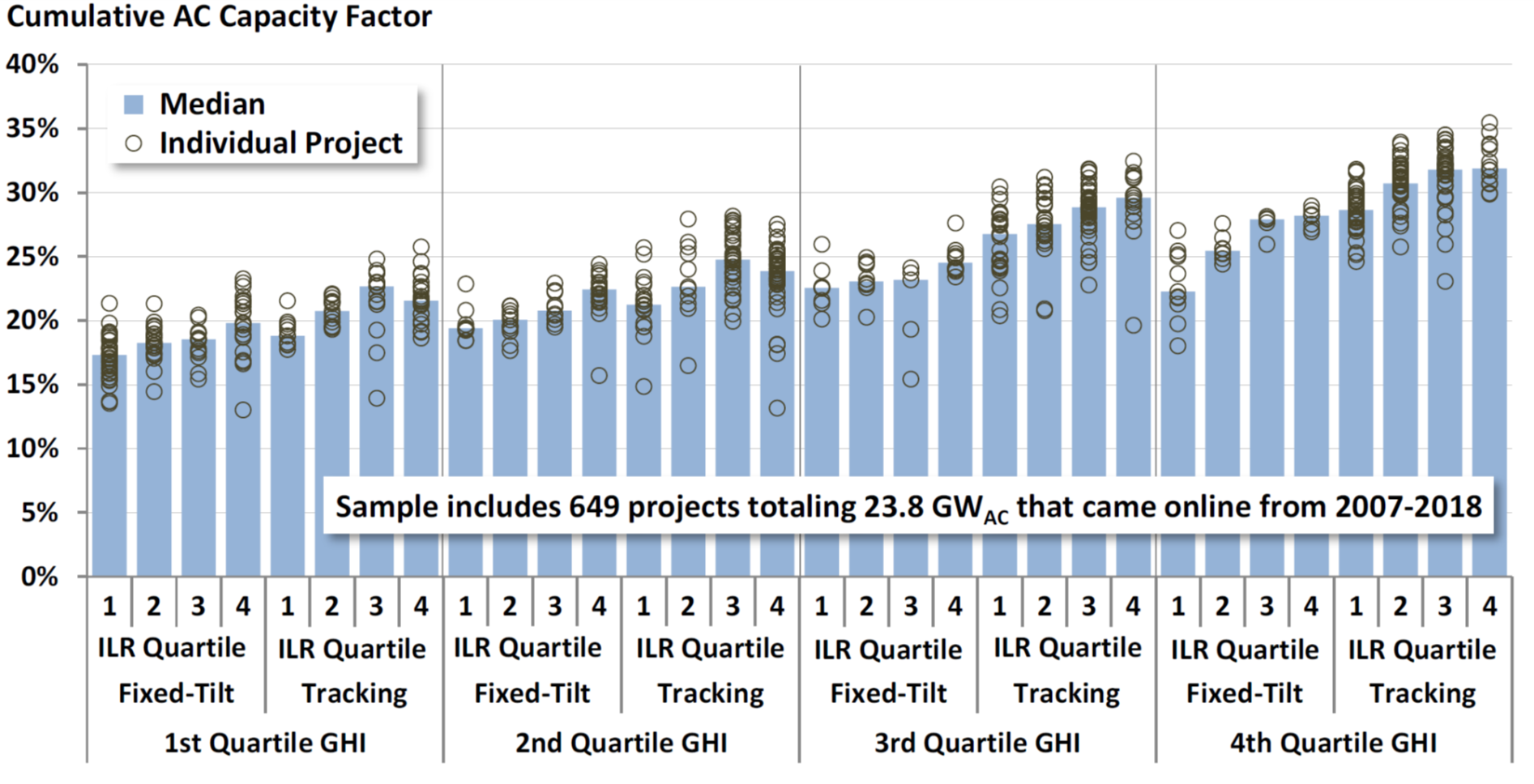
Source: (Bolinger et al., 2020)
Over time, PV plant output is reduced. This degradation (at 0.7%/yr) is accounted for in ATB estimates of capacity factor. The 2021 ATB capacity factor estimates represent estimated annual average energy production over a 30-year lifetime.
These AC capacity factors are for a one-axis tracking system with a DC-to-AC ratio of 1.34, and therefore is not representative of the lower capacity factors reported by fixed-tilt systems.
Base Year: In the interactive data chart at the top of this page, select Technology Detail = All to add filters to display a range of capacity factors based on variation in solar resource in the contiguous United States. The range of the Base Year estimates illustrate the effect of locating a utility-scale PV plant in places with lower or higher solar irradiance. The ATB provides the average capacity factor for 10 resource categories in the United States, binned by mean GHI. Average capacity factors are calculated using county-level capacity factor averages from the Renewable Energy Potential (reV) model for years 1998–2019 (inclusive) of the National Solar Radiation Database (NSRDB). The NSRDB provides modeled spatiotemporal solar irradiance resource data at 4-km spatial and 0.5-hour temporal resolution. The county-level mean GHI is calculated by aggregating each individual NSRDB point’s multiyear mean GHI to provide the county’s mean GHI for all years included in the analysis. U.S. average capacity factor for each resource category is weighted by the land area (square mileage) of each county within the GHI resource category. The county estimated land area is provided by geospatial and tabular data from the U.S. Census.
Because of the change in methodology in calculating capacity factors in the 2021 ATB, they are not directly comparable to previous editions of the ATB. In the 2021 ATB, we use capacity factors, ranging from 19.3% for Class 10 (for locations with an average annual GHI less than 3.75) to 30.9% for Class 1 (for locations with an average annual GHI greater than 5.75). The 2021 ATB capacity factor assumptions are based on ILR = 1.34.
Future Years: Projections of capacity factors for plants installed in future years increase over time because of an increase in energy yield from the module (better tracking, improved cell temperature, bifaciality, and better citing/practices to improve albedo), reduced system losses (improved soil removal, improved O&M uptime, and more-efficient inverters) and a reduction in degradation rates. The table below summarizes the technology improvements we used to calculate indicative improvements in capacity factor in each scenarios.
Performance Area | 2019 | 2030 Conservative | 2030 Moderate | 2030 Advanced |
---|---|---|---|---|
Bifaciality Factor | none | none | 0.85 | 0.85 |
Albedo | 0.2 | 0.2 | 0.2 | 0.3 |
AC and DC losses | 14.3% | 14.3% | 10.4% | 7.5% |
Annual degradation rate | 0.7% | 0.7% | 0.5% | 0.2% |
The technology improvements summarized above would not necessarily result in the estimated capacity factor improvements, given the 2021 ATB assumption of a constant ILR of 1.34. PV system ILR choice is based on an optimization exercise to maximize profits (or offer the lowest energy price), trading off the extra cost and increased clipping losses of additional modules with improvements in inverter operation and a higher, flatter electricity production curve. All things being equal, the optimal ILR of PV systems in higher resource classes or those which use bifacial modules will be lower than the optimal ILR of systems in lower resource classes or those with monofacial modules, particularly without the use of storage. Because of the complexity of optimizing CAPEX and ILR for each resource class for each year, and with and without storage, ATB PV system CAPEX and capacity factor benchmarks are calculated using a fixed ILR of 1.34, independent of system location, performance improvements over time, or the incorporation of storage. Additionally, we assume performance improvements over time are not location-dependent, despite the fact that a PV system with the same ILR in a higher resource area will experience more clipping, and thus lower performance improvements. However, in reality, PV systems in those areas would reduce their clipping losses by installing fewer PV panels and would thus have a lower upfront cost (trading off the marginally greater production with reduced CAPEX).
The following table summarizes the difference in average capacity factor in 2030 caused by these changes in the three technology innovation scenarios. Similar to our CAPEX assumptions, we assume each scenario's 2050 capacity factor is the equivalent of the 2030 capacity factor of the scenario but one degree more aggressive, with a straight-line change in price in the intermediate years between 2030 and 2050. The table below summarizes capacity factors for each ATB scenario by resource class.
Scenario | Lifetime Average Capacity (Class 10–Class 1) | Percentage Improvement from 2019 |
---|---|---|
Advanced Scenario | 23.1%–37.1% | 20.0% |
Moderate Scenario | 21.6%–34.6% | 11.9% |
Conservative Scenario | 19.3%–30.9% | 0% |
We also develop and model a scenario one scenario more aggressive than the Advanced Scenario to estimate capacity factor in 2050. In the Advanced Scenario, capacity factor in 2050 is assumed to have a 25% improvement over 2019 capacity factors.
References
The following references are specific to this page; for all references in this ATB, see References.